Respiration: Oxygen, gas exchange, and energy release
by Kenrick Vezina
Did you know more than 370 species of lungless salamander receive all their oxygen through their skin? Though you might be familiar with respiratory organs like lungs or gills, organisms obtain oxygen in various ways. But what makes oxygen so important?
Although the importance of breath has been recognized by many cultures throughout human history—for example, as with the Indian concept of prana—it wasn’t until experiments in the 1700s that oxygen gas was identified as the specific substance being consumed when we breathe.
Respiration is the process of extracting usable chemical energy from organic molecules, and it is performed by all living things; it can be thought of as a more controlled analogue to burning fuel for heat and light.
The combined work of biologists and physicists shows that the many structures organisms have evolved for gas exchange (e.g., lungs and gills) have been shaped by the need to maximize surface area for oxygen diffusion.
Oxygen that’s not used up in respiration quickly becomes toxic to living tissue. In recent years, some biologists have argued that this is why many insects exhibit strange patterns of opening and closing their respiratory systems: to limit their exposure to oxygen.
Adenosine triphosphate (ATP) is the primary product of respiration; once this was discovered, biochemists were able to precisely measure the difference in efficiency between aerobic and anaerobic respiration—a 19 to 1 difference.
- bacteria
- A large group of one-celled organisms found almost everywhere
- byproduct
- An unintended product resulting from the creation of something else
- cell
- The basic structural unit of all living things
- diffuse
- The movement of atoms from one part of a medium to another caused by their random thermal motion
- filament
- A thin, flexible threadlike appendage found in animals and plant structures
- molecule
- A particle formed from the bonding of two or more atoms
- net movement
- Occurs when molecules move from a region of higher concentration to a region of lower concentration due to random molecular motion.
- volume
- The amount of space taken up by matter
Introduction
Picture this: We’re in the late 18th-century France, and a shirtless young man sealed inside an airtight mask sits in a room lined with shelves of jars. Pipes connect him to a large reservoir, part of a room-spanning arrangement of chemistry equipment. A one-way valve ensures that his exhalations are collected through the pipes as he breathes.
French chemist Antoine Lavoisier is at the other end of the equipment, hunched over a basin. The man in the mask is his fellow scientist and collaborator, Armand Séguin. Several other men assist with the proceedings—one has his hand on Séguin’s wrist, tracking his pulse.
Meanwhile, Lavoisier’s wife, Marie-Anne Lavoisier, sits at a drawing table nearby, documenting the experiment through her careful illustrations (Figure 1). That we know as much as we do about this scene, and the rest of Lavoisier’s work, is thanks to Marie-Anne’s diligent, often uncredited, work as a scientific illustrator, record-keeper, and translator.
All the people in the room are working toward one goal: to measure how much “respirable air” Séguin consumes at rest.
Figure 1: Marie-Anne Lavoisier’s illustration of one of her husband’s experiments in respiration. Seated to the left is collaborator Armand Séguin. Lavoisier’s husband, Antoine Lavoisier, is leaning over the basin toward the right. And Lady Lavoisier herself can be seen at her drawing table on the far right. Séguin is connected to an apparatus to measure the amount of oxygen he consumes with each breath.
image © Public DomainThe “respirable air” Lavoisier was interested in had been identified earlier in the 1770s by two scientists working independently—Carl W. Scheele in Sweden and Joseph Priestley in England—had successfully isolated a gas that made candles burn faster than usual. After testing this gas on mice, Priestly tried breathing it himself and found that his “breast felt peculiarly light and easy" (Kean, 2017).
Not long later, Priestley and Lavoisier met at a dinner party. Inspired by this conversation, Lavoisier carried forward (or stole, depending on who you ask) Priestley’s work with this mystery gas. Séguin was recruited as a guinea pig and spent several sessions in the laboratory having his breathing rate, pulse, inhalations and exhalations chemically examined. In 1779, Lavoisier named the gas oxygen. (Read more about Priestley and Lavoisier’s contributions to chemistry in our Early Ideas about Matter module.)
In isolating the specific element living things need from the air they breathe, Lavoisier and his colleagues had achieved a major milestone in the study of respiration. But the questions of how and why our bodies absorb oxygen remained.
What is respiration?
We all recognize the importance of breathing from a very young age. Think of the first time you tried to hold your breath and were confronted with your body’s overwhelming urge to gasp for air.
In ancient India, breath (called prana) was thought to connect humankind to the cosmos, acting as an expression of a person’s immortal soul. Similarly, traditional Chinese medicine views breath as intimately related to one’s vital energy (called qi or sometimes ki or chi). And in the Stoic philosophy of Ancient Greece, the breath of life (called pneuma) was a force that structures both matter and the human soul. Meanwhile, modern medicine has vindicated the idea that deep, intentional breathing has whole-body health benefits, such as reducing stress and calming the nervous system.
Breathing—taking oxygen from the air and expelling carbon dioxide—is just one step in the “respiration” process, which spans the entire body. Respiration is how living things transform the energy contained in their food into a form usable by individual cells.
It’s important to note that not all forms of respiration actually require oxygen. Many bacteria, fungi, and other microorganisms don’t use oxygen in their respiratory process. Respiration without oxygen is called “anaerobic respiration,” while respiration in the presence of oxygen is called “aerobic respiration.” However, aerobic respiration is far more efficient in terms of the usable energy it produces per unit of fuel than anaerobic respiration. As a result, the development of aerobic respiration was a major milestone in the history of life on Earth. Without it, the evolution of complex and large-bodied plants and animals that require large amounts of energy to sustain themselves may not have been possible.
Comprehension Checkpoint
Respiration can be split into two major phases: “gas exchange” (of which breathing is a major step) and "cellular respiration.”
Gas exchange is the process of absorbing oxygen from the external environment and distributing it to the body’s cells, as well as the transport and elimination of carbon. Cellular respiration, on the other hand, is the complex series of chemical reactions that happen inside a cell, ultimately turning sugar into usable energy and releasing carbon dioxide and water as byproducts.
In this module, we’ll focus on gas exchange, while cellular respiration is covered in our modules Energy Metabolism I and Energy Metabolism II.
Direct diffusion, the simplest method of gas exchange
Although there are many forms of gas exchange, they all depend on the physical properties of fluids (gases and liquids). Because atoms and molecules in fluids are in constant motion, they tend to spread from areas of high concentration to areas of low concentration in a process called “diffusion.” The greater the difference in concentrations, the faster the overall diffusion of molecules takes place (see our Diffusion module).
Living things take advantage of diffusion by exposing a permeable (penetrable) surface to oxygen-rich fluids like air and water. Think about what would happen if you accidentally placed a book or magazine into a puddle of water. Over time, because paper is permeable to water, the liquid will seep into it. If you pick it up right away, only the bottom will be wet. Yet, if you leave it for an hour or more, the whole book might soak through. In this example, the water has diffused into the book’s pages.
As long as the oxygen concentration in the outside environment is higher than that inside an organism’s body, oxygen molecules will naturally flow across any permeable surfaces into the tissues. The greater the surface area exposed, the more quickly an organism can absorb oxygen. Because the path molecules move in is aimless and random, individual oxygen molecules will always be moving both into and out of the body. However, when there is a difference in the concentration, the net movement will be from the area of high concentration (e.g., air outside the body) to the area of lower concentration (e.g., tissues inside the body).
Currently, Earth’s atmosphere is about 21% gaseous oxygen. Oxygen concentrations in bodies of water on Earth vary, but well-oxygenated water on the ocean’s surface is about 0.495% dissolved oxygen. Microorganisms and some smaller or less active creatures, like flatworms, sponges, and jellyfish, can obtain enough oxygen from the atmosphere or ocean through “direct diffusion”—the passive movement of oxygen into their tissues through the external surfaces of their bodies. Direct diffusion is the simplest method of gas exchange, as it needs no specialized biological structures or behaviors, just an exterior surface that is at least somewhat permeable to oxygen.
Direct diffusion is not enough for large organisms
Because a marine flatworm has a very thin body, none of its cells are more than a few cell layers away from the outside environment. In contrast, an African elephant has a much larger amount of internal volume relative to its external surface. Some of its deeper tissues may be a full meter or more away from the air. This is because of the mathematical relationship between surface area and volume: If you increase the size of an object, its volume increases much more than its relative surface area.
Imagine a marine flatworm as a very thin rectangular box. Let’s say it’s ten millimeters long, five millimeters wide, and one millimeter tall. Let’s see what happens to its surface area, volume, and the ratio between them if you make it ten times longer or 100 times longer without changing its shape (see interactive animation). Note that the flatworm actually has a greater external surface area than volume at its natural size.
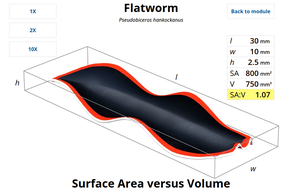
Interactive Animation: Volume versus surface area
Even if an elephant had very permeable skin (which it does not), it would be impossible for enough oxygen to diffuse deeply into its tissues. Diffusion tends to be a gradual process. Relying on diffusion alone to move oxygen through more than a few layers of cells takes too long to be useful across more than a few layers of cells. As a result, direct diffusion cannot meet the gas exchange needs of larger organisms.
One way to reduce the distance between internal tissues and the external atmosphere is to bring air directly into the body. This is exactly how insects get oxygen, using a “tracheal respiratory system.” Tiny holes in the insects’ exoskeletons, called “spiracles,” connect to narrow, branching tubes called “tracheae.” Air flows into these tubes and diffuses directly into the body tissues.
Scientists have been aware of the tracheal system’s essential function in insects at least since 1669 when Italian biologist and physician Marcello Malpighi published a detailed anatomical study of the silkworm moth and correctly grasped the tracheal system’s purpose.
Although an animal can have both a tracheal system and an oxygen-carrying circulatory system (as found in spiders and lobsters), insects do not. Insects have a circulatory system but lack true blood or vessels to contain it. Instead, they have “hemolymph,” a fluid that flows freely inside the spaces of the body, bathing the organs and tissues. Unlike blood, hemolymph does not efficiently transport oxygen around the body, so it is not an important component of insect respiration.
The surfaces of many plants’ leaves and stems contain similar openings for gas exchange called “stomata” and “lenticels,” respectively (Figure 2). Insects have several ways they can regulate gas exchange through their tracheal system, including opening and closing their spiracles like valves or flexing their muscles to force air in and out more efficiently. This brings fresh air, with its high oxygen content, deep into the insect’s body so that diffusion can do its work. In effect, it creates much more surface area for gas exchange.
Figure 2: Close-up photo of an insect spiracle, side-by-side with leaf stomata.
image © Left: CC BY 2.0 siamesepuppy; Right: Public DomainComprehension Checkpoint
The blood-based circulatory system: an oxygen-carrying superhighway
Insects’ tracheal respiratory systems are very efficient at small scales but cannot meet the challenge of moving oxygen around a large body. This is one of the primary reasons that all insects are relatively small.
The presence of red blood cells is one of the key differences between your blood and insect hemolymph. Red blood cells make human (and other vertebrates’) blood extremely good at carrying oxygen. More specifically, red blood cells contain a specialized oxygen-binding protein called “hemoglobin.”
Without a specialized carrier molecule like hemoglobin, oxygen dissolves poorly in “plasma,” the water-based substance that makes up the fluid portion of blood. Even well-oxygenated water contains less than 1% dissolved oxygen, compared to air’s 21%. This is because the chemical properties of oxygen gas and water make them incompatible at the molecular level (see our Chemical Bonding module).
However, each hemoglobin molecule can bond with four oxygen molecules, and there are 250 million hemoglobin molecules in a single red blood cell. That works out to a carrying capacity of approximately 1 billion oxygen molecules per red blood cell. As a result, hemoglobin carries 98% of the oxygen in your blood. Indeed, blood is so efficient at oxygen storage that concentrations of oxygen in blood may be more than 30 times higher than in an equivalent amount of water (Figure 3).
Figure 3: Bar chart showing approximate concentrations of oxygen in air, water, and blood, per table below. The 20% in blood may seem low relative to the 21% in air, but remember that blood is water-based, so it is more appropriately compared to seawater (<0.5%).
image ©VisionlearningOther groups of animals have also evolved different oxygen-carrying molecules than mammals. “Hemocyanin” is functionally like hemoglobin but is blue and found spiders’ and lobsters’ blood.
Even without specialized oxygen-absorbing organs like lungs or gills, a blood-based circulatory system greatly improves gas exchange efficiency. Several groups of non-insect invertebrates, like earthworms and leeches, also have blood-based circulatory systems. In fact, not all vertebrates have lungs. Although adult amphibians usually have lungs, they have lost their lungs at multiple points in their evolutionary history. There are currently more than 370 species of lungless salamanders that obtain their oxygen through their skin (especially the lining of their mouth and throat) and circulate it through their body with blood.
Biologists studying lungless salamanders have noticed physical and behavioral adaptations that would improve their respiratory abilities, including increased blood flow near the skin and pumping air in and out of their mouths by pulsing their throat muscles. In 2016, Harvard biologist James Hanken and his team decided to look for genetic evidence of adaptations for a lungless existence. Sure enough, genetic analysis of these salamanders found that they express a copy of a gene normally associated with lung function in their skin. This led Hanken and his team to conclude that lungless salamanders have repurposed a key protein for lung function to improve their ability to breathe through their skin.
Comprehension Checkpoint
Lungs and gills: specialized oxygen-absorbing organs
Larger and more active creatures have evolved complex oxygen-extracting organs to meet their gas exchange needs. Such organs work in close coordination with an oxygen-carrying circulatory system. This combination is very good at both extracting oxygen from air or water and moving it around the body quickly.
For aquatic animals like fish and the larvae of many amphibians, those oxygen-extracting organs are gills. For mammals, they are lungs. Compare the external gills of the axolotl, a permanently aquatic salamander, and the internal structure of human lungs (Figure 4). You may notice they look like inverse images of one another. This is no coincidence.
Lungs and gills operate on the same fundamental principle: maximizing the available surface area for gasses to diffuse across. Axolotl gills do this by branching outward into hundreds of feathery external filaments that maximize the surface area touching the oxygenated water. Human lungs consist of branching tubes and pockets to maximize the surface area exposed to the air.
Figure 4: Side-by-side images of an axolotl’s external gills and the branching structure inside a human lung.
image ©Left: CC BY 4.0 DataBase Center for Life Science (DBCLS); Right: CC BY 3.0 Adrien CrettéEstimates of the total internal surface area within human lungs vary from 70 m2 to 140 m2 (750 ft2 to 1500 ft2)—roughly equivalent to the floor space of a large one-bedroom apartment or a little less than half of a tennis court. In contrast, the standard estimate of the external surface area of an adult human (our skin) is only about 1.7 m2 (18 ft2).
Figure 5: side-by-side proportionate squares for surface area of an adult human vs surface area inside the lungs.
image ©VisionlearningIn both gills and lungs, the tissues are heavily packed with “capillaries,” tiny blood vessels that allow gasses to diffuse quickly in and out of the bloodstream. Remember when we mentioned diffusion only working quickly across very small distances? Capillaries are ten times thinner than an average sheet of paper. In fact, capillaries are so thin that red blood cells can only fit down them in a single-file line. Oxygen quickly diffuses across the cells of the capillaries and into the red blood cells, where it binds to an oxygen-carrying pigment.
Oxidative damage and the mystery of discontinuous breathing in insects
Until now, we’ve only explored the ways that organisms increase the amount of oxygen in their bodies. However, the same chemical properties that make oxygen useful to the process of respiration make it detrimental: Oxygen tends to produce extremely reactive particles that can damage living tissue. This is called “oxidative damage,” and it may be the key to an ongoing mystery in insect respiration biology.
In a 1955 report published in The Biological Bulletin, American zoologists Howard A. Schneiderman and Carroll M. Williams noted a “series of puzzling observations” made during their attempts to quantify the respiration of silkworm moth pupa. Schneiderman and Williams expected a smooth pattern of oxygen in and carbon dioxide out. Instead, they measured a constant rate of oxygen consumption and sudden, periodic spikes in carbon dioxide release followed by long stretches with virtually no carbon dioxide release (Figure 6).
Figure 6: Rates of oxygen consumption and carbon dioxide output of a diapausing pupa over an 18-hour interval.
image © Schneiderman & Williams, 1955The bursts of carbon dioxide release correspond to patterns in spiracle activity:
- A brief period during which the spiracles are fully open
- Another brief period during which they are fully closed
- A longer period of fluttering rapidly open and closed, resulting in constricted airflow
In the years since Schneiderman and Williams’s report, biologists have recorded a similar pattern in many other insects called “discontinuous gas exchange” or “discontinuous respiration.”
Exactly why insects do this is a topic of active investigation. Some scientists initially thought the spiracles closed to prevent water loss by minimizing exposure of moist internal tissues to dry air. Another possibility was that the insects needed to compensate for the effects of living underground, where poor ventilation could mean carbon dioxide concentrations become dangerously high over time.
Biologists Stefan K. Hetz and Timothy J. Bradley, working in the early 2000s, were unsatisfied with either argument. They felt these explanations overlooked the importance of oxidative damage. They knew, for example, that other scientists’ experiments showed that otherwise healthy fruit flies suffer oxidative damage to their tissues over time, even under normal conditions. Perhaps the trade-off between oxygen’s metabolic usefulness and its potential for harm is what drove the evolution of discontinuous respiration?
To test their hypothesis, Hetz and Bradley performed measurements on an atlas moth pupa, which has large and easy-to-access spiracles along the sides of its body. By using a variety of sensors, Hetz and Bradley measured a wide range of data during the different stages of discontinuous respiration, including:
- internal air pressure of the tracheal system
- concentration of oxygen in the tracheal system
- abdominal movements
- oxygen consumption and carbon dioxide release over time
They also experimentally altered the pupa’s environment, changing the oxygen concentration in the air around it to see how this affected its respiration. Even when they increased the oxygen concentration to more than twice the everyday amount, oxygen concentration inside the tracheal system remained nearly constant (Figure 7).
When the moth closes its spiracles, oxygen concentration decreases as the tissues absorb the oxygen and use it for respiration. However, because the rate of carbon dioxide (CO2) released from insect tissue is always slower than the rate of oxygen uptake, CO2 begins to build up. So the insect flutters its spiracles open and closed, allowing some CO2 to escape and a bit of oxygen to enter. It can sustain this breathing mode longer than if the spiracles were fully open or fully closed. Still, CO2 concentrations eventually reach a point where the insect must fully open its spiracles to vent the excess gas (Figure 7). When this happens, oxygen concentrations rise to dangerous levels, and the cycle begins anew.
Figure 7: Even when ambient oxygen concentration increases, the concentration of oxygen inside the trachea holds steady at a low level.
image © Hetz & Bradley, 2005Notably, insects that display discontinuous gas exchange only do so when at rest and in comfortable conditions. When exposed to metabolically demanding situations, such as times of high activity or high temperatures, insects leave their spiracles wide open. Therefore, Hetz and Bradley argue discontinuous breathing is a form of “idling.” Insects use this to keep from experiencing oxidative damage when their high-efficiency gas exchange systems do not need to run at full capacity.
This investigation underscores an essential fact of respiration: Every organism’s respiratory system has an upper limit on how quickly it can safely use oxygen. Remember that the earth’s atmosphere at sea level is only about 21% oxygen, and this is what our respiratory systems have adapted to. They work very well under these conditions. If you’re healthy, your respiratory system keeps your blood almost fully saturated with oxygen. However, breathing pure oxygen will quickly begin to cause irreparable lung damage.
Comprehension Checkpoint
Cellular respiration and the production of adenosine triphosphate (ATP)
As long as cells are supplied with an appropriate amount of oxygen and fuel molecules (i.e., food), they can perform their half of the respiration process, appropriately named “cellular respiration.” This process remained a mystery to Lavoisier and his peers in the 18th century. Still, Lavoisier suspected that oxygen might be involved in something like a controlled burning in the lungs, given its effects to an open flame.
The primary fuel for cellular respiration is “glucose,” a simple sugar obtained from broken-down food—although other biological molecules like fats and amino acids may also be used. Scientists had recognized glucose’s importance by the start of the 20th century. Yet, working out the chemical steps cells use to turn glucose into usable energy would take most of the next century.
The essential product of cellular respiration was unidentified until 1939, when German-American biochemist Fritz Lipmann demonstrated that the energy being released from glucose was being transferred into another molecule: adenosine triphosphate (ATP). This remarkable molecule acts as the energy currency of living cells by storing energy in its chemical structure. By creating or modifying this molecule, cells can store or release energy, with units of ATP acting like tiny molecular batteries. It’s moved around the cell as needed to power various chemical reactions that ultimately keep the cell alive.
The true efficiency of aerobic respiration and the corresponding importance of oxygen become obvious in terms of ATP. Through aerobic respiration, a single molecule of glucose yields 38 ATP molecules. In contrast, anaerobic respiration produces just two ATP molecules. That’s a nearly 20-times difference in efficiency! It’s little wonder that the quest for efficient gas exchange has shaped the biology of every multicellular organism on the planet.
Table of Contents
- Introduction
- What is respiration?
- Direct diffusion, the simplest method of gas exchange
- Direct diffusion is not enough for large organisms
- The blood-based circulatory system: an oxygen-carrying superhighway
- Lungs and gills: specialized oxygen-absorbing organs
- Oxidative damage and the mystery of discontinuous breathing in insects
- Cellular respiration and the production of adenosine triphosphate (ATP)
Activate glossary term highlighting to easily identify key terms within the module. Once highlighted, you can click on these terms to view their definitions.
Activate NGSS annotations to easily identify NGSS standards within the module. Once highlighted, you can click on them to view these standards.